Equipment¶
A typical patch clamp recording setup is shown in figure 2, and minimally consists of an amplifier, a microscope, a data acquisition system, a solution delivery system, and a chamber that holds the brain slice.
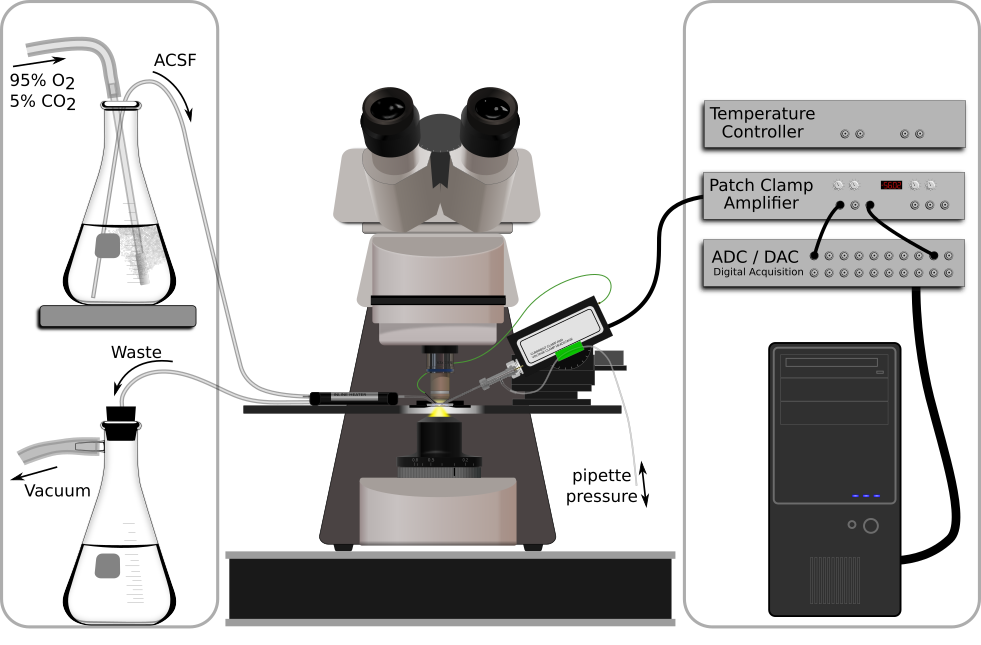
Figure 2. A minimal patch electrophysiology rig. Left to right: Oxygenated ACSF is siphoned through a fluid heater and into the recording chamber where it continuously washes over the brain slice. Fluid is then aspirated out of the recording chamber and into a waste flask. A patch clamp amplifier headstage is mounted to a micromanipulator and holds the patch pipette, which currently impales the brain slice (detailed in figure 3). The headstage ouput is amplified, digitized, and finally recorded on a computer.
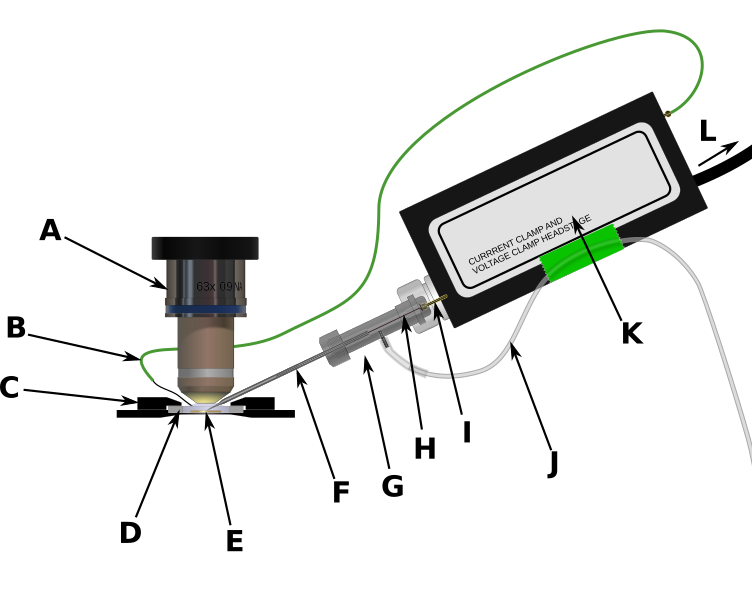
Figure 3. Patch recording equipment. A) 63x ceramic, water-immersion objective. B) Silver chloride wire connected to headstage ground output. C) Heated aluminum holder for recording chamber. D) Plastic recording chamber with glass coverslip on the bottom. E) Brain slice bathed in warm, oxygenated ACSF. F) Glass patch pipette filled with electrode solution. G) Electrode holder. H) AgCl electrode wire. This wire fits inside the patch pipette and makes electrical contact with the electrode solution as well as the I) gold pin which conducts electrode potential into the amplifier headstage. J) Pressure control tube. This allows the experimenter to increase or decrease the pressure inside the patch pipette. K) amplifier headstage.
Amplifiers¶
Currents in neurons span a wide range, from picoamperes (pA) for single channels and miniature synaptic currents, to tens of nA for currents through some voltage-gated ion channels. Similarly, voltages in neurons range from tens of μV for synaptic potentials to about 100 mV for action potentials. Consequently, specialized equipment is required to amplify the signals before they are recorded. The amplifiers used in electrophysiology are uniquely suited to recording such signals while introducing as little noise as possible.
Whereas early patch clampers had to build their amplifiers, a variety of commercial amplifiers are now available that have capabilities and specifications that would be hard for an individual laboratory to duplicate. The choice of an amplifier should be guided by the goals of the experiments, as not all amplifiers have the same merit figures for noise, or bandwidths, and some are better suited for particular experiments. The amplifiers consist of two main parts. The headstage is usually placed close to the recording site, and may hold the electrode directly. The headstage is connected to the main part of the amplifier, which provides the controls, input and output signals, and usually interfaces with a computer. The headstage also provides a high-quality ground output that is used to ground the fluid in the recording chamber and provide a clean reference for measuring potentials.
If the goal is to make current clamp recordings, a voltage-follower headstage is recommended. This type of headstage has a very high input impedance, low input capacitance, and a fairly wide bandwidth. Usually these headstages are also equipped with a current injection circuit, and circuitry that allows compensation of the electrode capacitance. As a result, they can faithfully report the voltage that appears at the input terminal, or at the wire that is inserted into the electrode. With properly prepared electrodes, the measured voltage can be nearly identical to the voltage at the tip, although it will be affected by electrode capacitance, which creates a low-pass filter that will attenuate rapid changes in voltage, as well as the voltage drop introduced by any current flowing through the electrode.
When the goal is to make voltage clamp recordings, the headstage is usually configured as a current-to-voltage converter. Because of the bandwidth limitations that arise when high gain is needed (for example, pA to V), the amplifiers include additional internal circuitry that helps to extend the bandwidth of the amplifier. Such headstages can also be operated in a mode that allows voltage measurement (as in current clamp), but limitations of the circuit can result in distortions of the voltage waveform (Magistretti et al., 1988).
Some modern amplifiers have dual-function headstages that provide very good performance in both voltage and current clamp, making it possible to collect data in both modes from individual cells, and even to switch between modes in the middle of a sweep. For example, this can be useful if you wish to elicit an action potential or a train of action potentials, and then measure the currents associated with the ensuing afterhyperpolarization.
Many modern amplifiers offer computer control, while older amplifiers were designed in an era when computers were used only to collect data from the amplifier. Having digital control of the amplifier and some internal signal processing, such as filtering, can be advantageous since it allows the experimenter greater flexibility and can simplify the execution of particular experimental manipulations. On the other hand the use of digital circuits, including digital signal processing, in the amplifier can introduce additional noise sources that can be difficult to eliminate and sometimes are hard to detect unless a wide-band oscilloscope is available. High frequency noise that is above the Nyquist frequency sampling limit imposed by the rate selected for the analog-to-digital (A/D) conversion process becomes folded down into lower frequencies, and adds to the apparent low frequency noise. (The Nyquist limit is one-half of the A/D conversion frequency, per channel. For example if you sample the voltage channel every 100 μsec, then the sample frequency is 10 kHz and the Nyquist limit is 5 kHz. All signals coming into the A/D converter should be low-pass filtered below 5 kHz. In practice, for 5 kHz filtering, the sampling rate should be at least 20 kHz.) We have observed high frequency noise on the output (following internal filtering) of a digitally controlled amplifier, and suggest that for recordings under conditions where low noise is demanded by the experimental measurements, analog amplifiers without internal digital processing may be preferable, or additional external filtering following the amplifier may be required.
Electrode holders¶
Properly functioning and clean electrode holders are critical to the success of patch clamp recording. The holders are typically made from a polycarbonate shell, and are designed to provide mechanical stability for the electrode, low capacitance, electrical insulation, and a low-noise connection to the input of the headstage (figure 3G). Holders should be cleaned regularly following the manufacturer’s instructions, and they should always be cleaned whenever the electrode solution gets into the holder.
The wire in the holder is usually made from silver and is coated with a thin chloride layer. The wire should be cleaned by carefully polishing with 600 grit polishing paper and washed with ethanol to remove any residue and oils. At this point, it is recommended to handle the wire with either tweezers or gloves. The chloride layer can be created by placing a cleaned wire into diluted bleach for a day or two. The last 1-2 mm of the wire should be cleaned to bare silver so that it can make good contact with the gold pin of the electrode holder (figure 3I). In some experiments, solutions are used which have a large electrochemical potential against silver-chloride, so in this case it is important to provide a bridge, usually made of agar, and 3M KCl to connect to the silver wire. Several published protocols can be found in the literature (Shao and Feldman, 2007; Snyder et al., 1999). Chlorided silver wires may also be used as the headstage ground wire, in lieu of commercially available ground wires with AgCl pellets attached.
Patching requires the application of negative and positive air pressure to the back of the pipette, so the holder will have a single port. We use a 1-2 cm length of silastic tubing to provide a flexible joint, and then ~20 cm of polyethelyene tubing to reach the table or the armrest on the isolation table (figure 3J). There are several methods of delivering air pressure. Some prefer to use a mouth-pipette, which provides excellent control over the pressure. We often use a 1cc tuberculin syringe (without the needle), and an adapter to connect to the tubing. With practice, the syringe can be used to make small changes in pressure that are either slow, for making seals, or fast, for rupturing the cell membrane.
When tightening the holder onto the headstage, it is often important to be careful to not make the holder too tight, as relaxation of the teflon coupler over time can introduce slow movement of the electrode. Similarly, the rubber retaining ring in most holders should not have much pressure on it when the cap is tightened, so that it does not relax and twist the electrode. A gentle, but firm finger tightening is sufficient.
Also, there is usually a gasket that seals access to the AgCl electrode at the back of the holder. This gasket is important to provide a pneumatic seal, and to keep fluid out of the connection to the headstage.
Microscope¶
Patch clamp recordings in brain slices are most successful when the cells to be recorded can be directly visualized, although “blind” patching is a technique that can be used under some circumstances. Direct visualization is usually achieved by using a fixed-stage, upright microscope with electrically insulating (for example, ceramic) water immersion objectives. The fixed microscope stage is often replaced with a fixed platform or a set of gantry towers that are fixed to the vibration isolation table, and the microscope placed on a translatable platform. An alternate approach is to fix the microscope to the vibration isolation table, and to have a translatable stage to hold the preparation and manipulators. This latter approach is commonly used when introducing laser light into the microscope, for example for 2-photon microscopy, since the optical platform must remain well aligned with the light source. It is usually not necessary to have more than 2 objectives on these microscopes. A low magnification (2.5-5X) long-working distance objective can be used to select the region of the slice and find the electrode for coarse positioning, while a high magnification (40X or 63X) 2-3 mm working distance water immersion objective is needed for visualizing and patching cells. It is best if the objectives can be exchanged and returned to the same focal position without requiring refocusing the microscope, and this is achieved with a sliding objective positioner. However, it is also possible to use a rotary objective turret, although this will require refocusing for each change of objective.
For young tissue or thin preparations, the use of Nomarski differential interference contrast optics (DIC) can help with the visualization of cell membranes and fine processes such as dendrites. In addition, the use of infrared (IR) or long-wavelength illumination reduces light scattering, and can be used to gain better visualization into deeper regions of the tissue. However, these long wavelengths also require a camera with good infrared sensitivity (usually a CCD camera) to actually visualize the preparation. Some CCD cameras have IR filters in front of the detector that reduce their natural IR sensitivity, and these have to be removed and replaced with an appropriate IR-transmitting filter.
For older, thicker or more heavily myelinated tissue, the use of DIC optics has little advantage, since the light polarization is partially randomized by the tissue. In this case, asymmetric or gradient illumination, followed by appropriate adjustments in image contrast on the monitor, works nearly as well. With modern cameras that have dynamic ranges of 12 or 16 bits, the contrast can be greatly increased around a mean level, allowing visualization of details that would be otherwise lost. The asymmetric or gradient illumination also helps increase the contrast. In the simplest case, such illumination can be obtained by adjusting the condenser off center from the light path, or by using a high-power IR light-emitting diode placed below the preparation and off the optical axis. We have used simple asymmetric illumination and image contrast adjustments to perform visualized patch recordings from neurons in 300 μm thick slices of adult mouse (> 80 days old) brainstem nuclei that have heavy myelination. In some cases, having software or hardware adjustments that allow the displayed image contrast to be enhanced, while subtracting background light levels, can also help visualize cells.
Blind patching is a technique whereby the patching is done without direct visualization (Blanton et al., 1989). In this case, the only feedback available is the electrical signal from the electrode. Blind patching can be done in vivo, or in thick tissues where no visualization is possible. However, the success rate is lower than for visualized patching.
Several approaches that fundamentally consist of optical workbenches with objectives and reconfigurable mechanical arrangements are now available. These may be preferable in some situations as they allow the rig to be changed to meet the demands of specific experiments or new optical configurations much more easily than if a dedicated microscope with enclosed optics is used.
Manipulators¶
Positioning the electrode requires the use of manipulators that allow smooth motion in 3 axes at the sub-micrometer level. Typically, this is achieved with mechanical, hydraulic, piezoelectric, or stepping motor manipulators. Each type has some advantages and disadvantages, but the current trend is towards piezoelectric and stepping-motor driven manipulators that have remote control units so that moving the electrode does not require touching the manipulator itself. The manipulators should be mounted securely on the same platform as the recording chamber. These manipulators will also often have a mechanical arrangement that allows the headstage and electrode holder to be easily brought out from under the objective to change electrodes.
Vibration Isolation¶
An important component of any patch clamp setup is reduction of building vibration. Most buildings have vibration that arises from air handling systems and nearby roadways (or railroad tracks), as well as foot traffic in the hall. Vibration that is transmitted to the electrode can make patching difficult or impossible. For patch clamp recording, tables can vary in size, although we typically use 30x48” tables with 4” deep tops to allow sufficient room for the microscope, light sources and ancillary equipment that is on the table. Smaller tables can be used as well, if they are located in an area with less vibration. Larger tables are only needed if there will be additional optics, such as lasers, on the table. The tables are “floated” using nitrogen supplied through a regulator. House air systems can be used if they have sufficient pressure, but it is recommended to provide an air filter and a water trap in the system to avoid mishaps that could damage the table.
Other hardware¶
Stimulators: One of the most common ways to activate pathways in a brain slice is to electrically stimulate the tissue using a bipolar or concentric electrode, usually no more than 250 μm in diameter. Simple stimulating electrodes can be made by twisting small gauge (22-30 ga) teflon-coated platinum wire together, cutting the ends flush with a sharp razor, attaching it to a twisted pair of wires that go to the stimulator, and inserting the platinum end through a fire-polished Pasteur pipette until the ends stick out of the pipette. A small drop of glue at the end of the pipette will help hold the wires in place. Commercial electrodes are also available in a variety of sizes and configurations from several vendors. The basic requirement for the stimulator is that the current (or voltage) and pulse duration be controlled. Typical pulse durations are 0.05 to 0.2 msec per stimulus. Voltages range from <1 to ~100 V, or if using constant current pulses, from 10’s of μA up to about 1 mA. Stimulus parameters are highly dependent upon the tissue type as well as the electrode configuration. The stimulator hardware consists of the pulse generator (this can be a computer or a stand-alone unit), along with an isolation unit that drives the electrode through a circuit that is electrically isolated from the rest of the setup. This isolation occurs either through an optical coupler, or a transformer. The output of the isolation unit should not be grounded.
Pipette puller: The preparation of the patch pipettes requires a puller suited to the purpose. Modern pullers are microprocessor-based devices that can create a pair of patch pipettes from glass blanks by heating the glass with either a filament or a laser, and cooling the glass with a jet of air. The choice of puller is not critical, as long as it is easy to modify the pulling pattern of heating and cooling and force. With some pullers it may also be necessary to have a microforge to fire-polish the tips of the electrodes. We have not found this necessary with a laser puller.
Slicers: The preparation of brain slices requires a slicer. To minimize damage to the tissue, slicers that use a vibrating blade that can be advanced through the tissue with a controlled rate, oscillation speed and distance, and angle, seem to work best. The slicer should be dedicated to brain slice preparation, as contamination with fixative or chemicals that might be encountered during histological processing is not conducive to the preparation of healthy, living brain slices.
Water filtration system: It is extremely important to have high quality water when preparing solutions for brain slices and patch clamp recordings. Contamination of the water used to make solutions by water treatment chemicals, bacteria, or various ions and salts that may be accumulated along the way, can lead to unexpected results and complications. The type of filtration system that is needed depends on the quality of water that is available to feed the system. For example, if your building provides reverse-osmosis treated water to each lab, then the system can be limited to the filter components needed to polish the water to a high quality. However, if you only have utility supplied water, you may need a reverse osmosis unit to generate a local supply that can be used to feed the polishing system. In our opinion, simple steam distillation of water is not sufficient. In addition, the water should be filtered with a 0.22 μm tissue-culture grade filter at the last step prior to use. It is also possible to purchase water, although this would be an expensive option.